The Earth wasn't always a friendly place to live. Not only was it covered in lava and constantly eruption, its atmosphere was chocked with volcanic gases like carbon dioxide and sulfur dioxide. How and when did the atmosphere reach its current oxygen-rich state? This post will walk through the processes as well as some of the evidence that allows us to understand what happened. While I describe as a series of apparently discrete steps, it's important to remember that these processes sometimes overlapped and that they occurred over a span of time, often millions or billions of years.
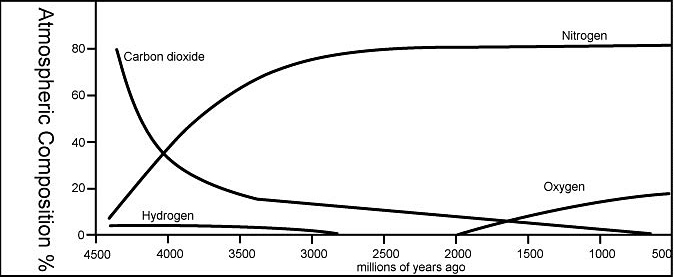
Image credit: Scotese
First Atmosphere: Totally Nebular, Dude
- Hydrogen (H2)
- Helium (He)
Immediately following Earth's formation, its atmospheric gases probably mirrored which elements are most abundant in the universe. Hydrogen, followed by helium, are by far the most common elements in the universe—and they're also the smallest. Helium and hydrogen are very light and they move rapidly when heated; powered by solar winds and perhaps the primordial Earth's own searing heat, hydrogen and helium escaped Earth's gravity. Much of it ended up at the edges of our solar system, gathered in Gas Giants like Jupiter.
Magnetosphere
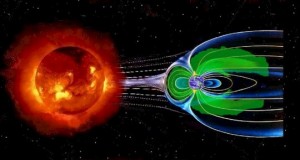
Magnetosphere. Image credit: NASA Heliophysics
Earth's metallic liquid outer core spins around its metallic solid inner core, generating an immense magnetic field that envelops the Earth and shields it from deadly solar winds. The magnetosphere is made possible by Earth's differentiation—that is, its distinct layers; immediately after the early formed 4.5 billion years ago, the layers were poorly developed and so the magnetosphere was extremely weak. The fledgling magnetosphere allowed solar winds to strip away the hydrogen and helium. Even after Earth had existed for a billion years (3.5 bya), the magnetosphere was still only half as strong as it is today.
Earth's robust magnetosphere is crucial to preserving our modern atmosphere. Without its heroic efforts, our precious oxygen would probably be blasted away—and as for us, we would be left to bake under a constant barrage of brutal solar winds.
Second Atmosphere: Volcanoes' Breath
- Water vapor (H2O)
- Carbon dioxide (CO2)
- Sulfur dioxide (SO2)
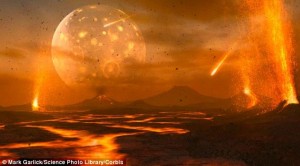
Image credit: Mark Garlick
After most of the hydrogen and helium blew away, what took over? Based on igneous and metamorphic deposits and reconstructions of Earth's internal temperature, we know that the early Earth was very hot and volcanically active, much more than today. Early Earth was so hot because of accretional heating; it had just formed from thousands of asteroids colliding together, and their kinetic energy transformed into heat. We also know from studying modern eruptions that volcanoes release a lot of gases (a process called volcanic outgassing), mostly likely the same kinds that ancient volcanoes expelled.
Volcanoes release different gases depending on the type of volcano (hot spot vs. subduction, for example) and the eruption temperature. However, almost without fail, the dominant gases, in order, are water, carbon dioxide, and sulfur dioxide. Small amounts of other gases, like carbon monoxide (CO), nitrogen (N2), ammonia (NH3), hydrogen chloride (HCl),and methane (CH4) also make regular appearances. With this information, we can use gases from modern volcanoes to infer what the early Earth looked like; with a haze of carbon dioxide and sulfur dioxide and with no free oxygen, it didn't look too friendly for mammals.
Although it makes intuitive sense to modern volcanic gases to reconstruct the ancient atmosphere, we can further confirm the approach's validity by calculating mass balances of volatiles. Atmospheric gases interact with the environment, so we can:
- Calculate the amounts of volcanic gases that are dissolved into modern surface reservoir.
- Calculate the pressures that these oxides would exert in gas form.
- Calculate what percent of the total pressure each oxide represents.
- Compare the calculated percents with the composition of gases released by volcanoes.
Volatile | Modern Surface Budget | Volcanic Gas Composition | |||
Major Reservoir | Amount (1023 g) | Pressure (bars) | Percent | Percent | |
H2O | oceans | 15 | 270 | 83% | 40-85% |
CO2 | sedimentary rocks | 0.75 | 53 | 53 | 2-50% |
SO2 | ocean | 0.13 | 5 | 1.5% | 1-15% |
N2 | atmosphere | 0.06 | 1.2 | 0.3% | <1% |
As you can see, the composition of modern volcanic gases matches nicely with our calculations for of ancient atmosphere.
The Fate of Volcanic Gases
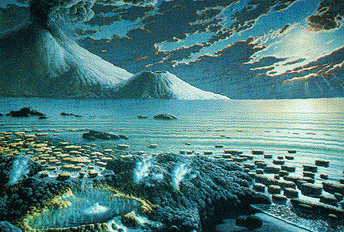
Volcanic outgassing of water vapor causes oceans to form. Image credit: Peter Sawyer, Smithsonian Institution
How do we go from an ancient atmosphere of mostly water and carbon dioxide to a modern atmosphere that has little of each? How did nitrogen, which amounts to less than one percent of volcanic gas, come to constitute a majority of our atmosphere?
The Losers
- As the Earth cooled, H2O vapor condensed into liquid, developing oceans.
- As oceans developed, CO2 and HCl dissolved into the ocean (and still do).
- NH3 was (and is) broken down by sunlight into N2 and H2.
- SO2 was (and is) recycled into the mantle.
The Winners: Nitrogen
- N2 stayed in the atmosphere!
Nitrogen prefers to stay in gas form, does not react with common Earth materials, and is stable in the presence of solar radiation, so nitrogen gas accumulated until it became the most abundant gas in the atmosphere.
Third Atmosphere: The Great Oxygenation Event
Modern Atmosphere
- Nitrogen (N2): 78%
- Oxygen (O2): 21%
- Carbon dioxide (CO2): 0.04%
- Water vapor (H2O): 0-4%
Between 2.3-2.5 billion years ago, the Great Oxygenation Event saw atmospheric levels of oxygen rise dramatically. Photosynthesis and tectonics had colluded to make conditions just right for the production and accumulation of free oxygen.
By the Phanerozoic (542 million years ago), oxygen reached modern levels with the help of a new evolutionary invention—land plants. Over the past 500 million years, oxygen levels have fluctuated up and down, hitting a high of 35% in the Carboniferous; today they stand at 21%.
Photosynthesis
The earliest photosynthetic organisms we know of are the cyanobacteria that left behind stromatolites as monuments to their planet-changing work. The oldest discovered stromatolites are 3.5 billion years old. Cyanobacteria build stromatolites by sticking sediments together to create a protective mat. Cyanobacteria need sunlight in order to photosynthesize, so when the overlying mat gets too thick, they move to the top and the cycle repeats, generating layered structures as the mats stack on top of each other. Stromatolites are still forming today in shallow, calm waters in Yucatan, the Bahamas, and Australia.

Stromatolite formation and fossilization. Image credits (left to right): James Golden, Aleksey Nagovitsyn, American Society for Microbiology, Paul Harrison, Noah Wild Blog
However, based on carbon inclusions in garnets from In western Greenland's Isua Complex, the first evidence of photosynthesis could date back as far as 3.8 billion years ago. Carbon-12 forms weaker bonds than carbon-13 and therefore requires less energy for organisms to work with, so life prefers carbon-12. If we find higher amounts of 12C than would otherwise naturally occur, it suggests that life was around. However, metamorphic processes can also alter carbon isotope ratios, so it is also possible that the inclusions' 13C/12C are not biological in origin.
In any case, at some point over a billion years before the Great Oxygenation Event, photosynthesizers began their important task of generating oxygen (and they haven't stopped since). So why didn't the Great Oxygenation Event coincide with the spread of photosynthesis? The mere appearance of photosynthetic organisms wasn't enough to cause the atmosphere to begin accumulating oxygen. For that to happen, it would take tectonics.
carbon dioxide + water ⇌ carbohydrate + oxygen
6CO2 + 6H2O ⇌ C6H12O6 + 6O2
Tectonics
As shown in the photosynthesis equation above, plants use energy supplied by sunlight to convert carbon dioxide and water into sugars and oxygen. However, the reaction is reversible: if the organic material is exposed to oxygen, the oxygen will react with the carbohydrates, leaving behind water and carbon dioxide. Without a way to keep oxygen and organic material separated, the reaction would continue back and forth until equilibrium was reached—which could have left us with much less oxygen than we're accustomed to. As oxygen-breathers, we want to keep the reaction heading toward the right. This is where tectonics comes into play.
Tectonics creates basins where organic material can buried and stored out of oxygen's reach. By the end of the Archean, most (90%) of the continents had formed, but Archean rocks (which are mostly igneous and metamorphic) are very hard to come by as they have been buried, metamorphosed, or subducted. However, in the Proterozoic—when the Great Oxidation Event occurred—extensive sedimentary basins appear in the rock record. Additionally, continental erosion created shallow seas that expanded the habitat for oxygen-producing stromatolites. Finally, some of the carbon dissolved in the ocean would be subducted into the mantle. In these ways, tectonics helped make conditions right for oxygen to accumulate.
Some Geological Evidence
We know that volcanoes were contributing to the atmosphere, but how can we be sure that there was no free oxygen? How do we know exactly when oxygen appeared?
Some of the most important pieces of evidence are:
- Banded Iron Formations (BIFs)
- Red Beds
- Pyrite Pebbles
- Sulfide Isotopes
Banded Iron Formations (BIFs)
Sources of Iron
Volcanoes and black smokers release iron, and during the hot Archean era, Earth had no short supply of eruptions. Over time, large amounts of iron accumulated in the oceans as volcanic deposits dissolved into seawater and black smokers pumped iron out of the seafloor.
Quick Chemistry
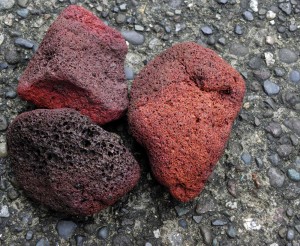
Volcanic rocks are rich in iron. Image credit: Jan Messersmith
Most atoms prefer to have eight valence electrons (the electrons in the outermost shell), so they will lose or gain electrons to reach that number. If an atom gains an electron, it becomes more negatively charged (reduced); if it loses an electron, it becomes more positively charged (oxidized).
Oxygen atoms have six valence electrons in their neutral state, so they like to gain two electrons, which gives the atoms an overall charge of -2.
O0 + 2e- → O2-
Neutral iron atoms can become more stable by losing electrons, which gives the atoms an overall positive charge. As transition metals, irons can lose a variable amount of electrons according to what works best at the time. The two most common charges are +2 and +3.
Fe0 - 2e- → Fe2+
Fe0 - 3e- → Fe3+
Neutral iron atoms and Fe2+ ions can be dissolved in seawater, but once Fe3+ ions will immediately react with oxygen and precipitate out.
Banded Iron Formations
When stromatolites appeared and began releasing free oxygen into seawater, the oxygen immediately reacted with the iron in seawater and caused the iron to precipitate as iron oxide minerals.
3O2- + 2Fe3+ → Fe2O3 (hematite)
3O2- + 1Fe2+ + 2Fe3+ → Fe3O4 (magnetite)
These deposits formed thick rock layers with alternating bands of red and black. The red layers are jasper, a form of silica (SiO2) with iron (Fe3+) impurities that gives it a red color. The dark layers are magnetite (Fe3O4), a magnetic iron oxide mineral. We still don't know exactly why the jasper and magnetite layers alternate, but it could have been driven by seasonal variations.
The stromatolites kept pumping oxygen, far outpacing the supply of iron (remember, as the Earth continued to cool, volcanism calmed down). Eventually, the ocean ran out of iron to react with oxygen, allowing the oxygen to escape into the atmosphere. Today, the ocean is full of oxygen, causing iron that reaches the ocean to instantly precipitate; therefore, iron deposits tend to be localized around black smokers.
Pyrite Pebbles
Pyrite (fool's gold), an iron sulfide mineral that has a chemical formula of FeS2, will form beautiful golden cubes in low oxygen environments, but it is highly unstable in the presence of oxygen. If oxygen is around, the sulfur ion readily reacts to form sulfate, leaving behind iron ions that then themselves react with oxygen. The Fe3+ then reacts with remaining pyrite, setting up a snowball effect whereby oxygen and Fe3+ iron work in unison to eat up pyrite.
2FeS2 (s) + 7O2 (g) + 2H2O (l) → 2Fe2+ (aq) + 4SO42- (aq) + 4H+
4Fe2+ (aq) + O2 (g) + 4H+ (aq) → 4Fe3+ (aq) + 2H2O (l)
FeS2 (s) + 14Fe3+ (aq) + 8H2O (l) → 15Fe2+ (aq) + 2SO42- (aq) + 16H+ (aq)
The ultimate effect of oxygen on pyrite is to "rust" it into hematite.
3O2 + 4FeS2 (pyrite) → Fe2O3 (hematite)
Therefore, the presence of pyrite in terrestrial rocks indicates that pyrite was stable under terrestrial conditions, meaning that the atmosphere had to be low in free oxygen—because if there was plentiful oxygen, the pyrite would break down.
In rocks between 3.8-2.4 billion years old, pyrite is plentiful as cement holding rock grains together and as rounded pebbles. Rounding of clasts indicates transport: in order to get rounded off, a clast must be moved long distances by wind or water. Therefore, rounded pyrite clasts suggests that pyrite could be exposed to surface conditions without reacting, and this means that there had to be no free oxygen.
Pyrite disappeared from terrestrial sediments and rocks 2.4 billion years ago when the Great Oxygenation Event is believed to have occurred. After the Great Oxygenation Event, there was just too much free oxygen to allow pyrite to form. Pyrite no longer forms at the surface.
Red Beds
Red beds are sedimentary rocks (usually sandstone, siltstone, and shale) that have a red color due to the presence of iron oxides. Before they reacted with oxygen, however, the beds would have had a different color. The appearance of oxygen caused the iron in iron-bearing silicate minerals to rust out into hematite, much like what happens to pyrite in the presence of oxygen.
Fe2SiO4 (olivine) + O2 → Fe2O3 (hematite) + SiO2 (quartz)
Red beds begin popping up not long after the Great Oxygenation Event, about 2.3 billion years ago. They didn't form before this because oxygen wasn't around to give the rocks their red color.
Summary
Earth's magnetosphere allows us to have an atmosphere. Early Earth had a low-oxygen atmosphere created by volcanic gases. Photosynthetic creatures appeared over 3.5 billion years ago and began creating free oxygen. Once the oxygen had reacted with all of the available iron in rocks and seawater, and sedimentary basins buried organic material so it couldn't react with the oxygen, free oxygen began accumulating in the atmosphere. During the Great Oxygenation Event 2.8 billion years ago, oxygen levels rose rapidly. Geological evidence includes banded iron formations, red beds, and terrestrial pyrite. At the start of the Proterozoic, oxygen in the atmosphere had reached modern levels.
Links
- An awesome interactive animation of oxygen levels over time
- An easy, bullet-point timeline of the evolution of Earth's atmosphere
- Wikipedia's section on the development of Earth's atmosphere
- A slideshow overview of pre-Cambrian Earth
- Idiot proof: a very simple NASA article for kids